Organic Aerosols in the Atmosphere
Atmospheric aerosols refer to the liquid or solid “clumps” of molecules floating through the ambient air. Typical sizes of aerosol particles range from several nanometers to several micrometers. They take a long time to settle to the ground because of the negligible gravitational pull relative to the Brownian motion forces exerted by random collisions with the gas-phase molecules and the forces originating from convective air currents. Aerosols play an important role in controlling the climate, driving atmospheric chemistry, and contributing to air pollution problems worldwide. For example, every single cloud or fog droplet requires a seed aerosol particle in order to grow. Atmospheric aerosols have received a lot of scientific attention in recent years because of the profound effects they have on the climate. Through multiple field observations, scientists have established that ambient particles contain a significant fraction of organic compounds. Particles that are dominated by the organic material are loosely defined as “organic aerosols” (OA), and further divided into primary and secondary by the mechanism of their generation. Primary Organic Aerosols (POA) are emitted in the atmosphere directly by various sources such as traffic, waves breaking, wind-blown soil, biomass burning, cooking, and so on. The molecular make-up of POA usually reflects the environment they came from. Secondary Organic Aerosols (SOA) are produced in the atmosphere as a result of complex chains of reactions that start with the oxidation of Volatile Organic Compounds (VOCs) by ozone (O3), hydroxyl radical (OH) and nitrate radical (NO3) and end with the condensation and coagulation of low-volatility oxidation products into aerosol particles. The presence of nitrogen oxides (NOx), an important component of urban pollution affects the formation of the aerosols. One especially important group of VOC that efficiently form SOA is terpenes, a class of hydrocarbons emitted predominantly by tree foliage. Terpenes include isoprene (C5H8), monoterpenes (C10H16) and larger terpenes.
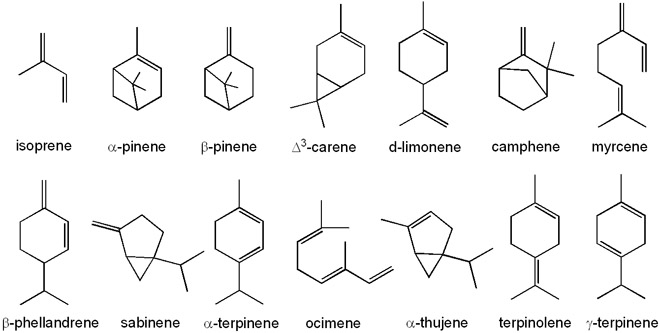
Imagine entering a garage in a house that has been occupied by a disorganized family for years. You will likely find dozens of dusty boxes filled with thousands of items that the family members used in the past but are now only vaguely aware of. Each particle in an organic aerosol is similar to such a garage in that it contains a bewildering number of products of atmospheric oxidation of various organic molecules, which were discarded by the air because they were not volatile enough to remain in the vapor state. Identifying each molecule in an organic aerosol is far more challenging then sorting through the mess of an old garage. However, it is worth doing this because detailed knowledge of molecular composition of organic particles holds clues to their important properties. For example, if we knew what kinds of molecules particles contain, we could predict what types of effects they may have on our health when we inhale them.
Even SOA generated from a single VOC under controlled laboratory conditions may contain thousands of different organic compounds. The real-world SOA are even more complicated than that. In collaboration with Prof. Alexander Laskin and Prof. Julia Laskin at Purdue University (formerly at EMSL, PNNL), we have been developing and applying methods of high resolution mass spectrometry (HRMS) to characterize the molecular composition of SOA [68]. This approach provides an unprecedented amount of detail about the composition of organic aerosols by supplying molecular formulas for thousands of aerosol species in a single measurement. Detailed analyses of the distribution of chemical formulas reveal useful mechanistic information about the chemistry leading to the initial formation and subsequent aging of biogenic and anthropogenic organic aerosols. The following figure is a highlight of our study of the composition of isoprene/ozone aerosol [64]; it displays a map of the O:C versus H:C ratios for about 1000 organic molecules that we were able to observe in this type of aerosol.
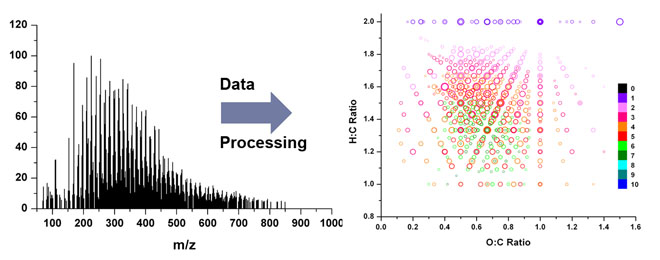
Molecular Composition of Secondary Organic Aerosols
The collaboration between our and Purdue University research groups has been really productive and it has resulted in the observation of multifunctional monomeric and oligomeric aerosol compounds in SOA prepared by oxidation of limonene [51, 56, 61, 66, 67, 69, 84, 173], isoprene [64, 70, 71, 75, 79, 80], alpha-pinene [99, 118], alpha-humulene [118, 153], toluene [125], naphthalene [93, 187], indole [119,184], beta-caryophyllene [163], farnesene [176], catechol [195], and other VOCs [100, 118]. In addition, we have examined composition of SOA prepared by oxidation of mixtures of VOCs, such as diesel fuel [116], mixtures simulating emissions from healthy and aphid-stressed trees [164], mixtures of d-limonene and beta-myrcene [194], etc.
In addition to examining lab-generated SOA, we have also analyzed ambient particulate matter samples collected in urban [96] and rural [85, 127] areas. For example, in collaboration with the group of Prof. Allen Goldstein from UC Berkeley, we analyzed aerosol samples from the 2010 CalNex field study in Bakersfield and Pasadena using positive mode nanospray-desorption electrospray ionization mass spectrometry [85]. That study represented the first systematic comparison between high-resolution mass spectra of field and chamber generated aerosols, and demonstrated that diesel was a major source of SOA in Bakersfield.
Molecular Composition of Biomass and Urban Material Burning Organic Aerosols
In collaboration with our colleagues from Purdue University and other research groups, we have examined chemical composition of POA from biomass burning organic aerosol (BBOA) [67, 114, 123, 127, 138, 151]. For example, we collected BBOA and quantified emission factors for particulate matter from cookstoves used in villages in rural India [157]. A more detailed analysis has resulted in a molecular inventory of particle-phase [127] and gaseous [139] species found in cookstove emissions, which were used in a modelling study to predict a large effect of cooking emissions on air quality in India [144]. We participated in the National Oceanic and Atmospheric Administration’s (NOAA) FIREX campaign. Forest fire fuels collected from around the country were burned during the 2016 fire lab intensive in Missoula, MT. In publication [151], we provided a comprehensive classification of light-absorbing BBOA organics based on fuel and combustion conditions, and observed lignin-derived products, distillation products, nitroaromatics, and polycyclic aromatic hydrocarbons.
We recently started a new NOAA-supported project to investigate properties of smoke from urban fires, in which both houses and vegetation burn. The frequency of fires occurring at the wildland–urban interface (WUI) is increasing, with biomass and a wide range of human-engineered materials serving as fuels. Urban material combustion is an important source of atmospheric particulate matter emissions. We used advanced analytical methods for molecular characterization of WUI smoke to identify both chromophoric and non-absorbing compounds in WUI smoke and confirm their structures using reference standards [200]. We are continuing this work with additional types of urban materials, as well as different burning conditions.
Linking Chemical Composition to Important Properties of Organic Aerosols
We have combined our group’s extensive expertise in the molecular level analysis of SOA with the unique methods of Prof. Allan Bertram's group at the University of British Columbia to measure and model rheological properties of SOA particles. These collaborative experiments have provided critically important data for refining versatile parametrizations that predict volatility and viscosity of organic particles from high resolution mass spectrometry data, developed by Prof. Manabu Shiraiwa’s group to reliably predict volatility and viscosity [131] distribution from high resolution mass spectrometry data. This greatly improves the utility of molecular composition data for atmospheric modeling.
In publication [150], we examined liquid–liquid phase separation (LLPS) and viscosities within SOA generated by the photooxidation of diesel fuel vapors, a good proxy for SOA from anthropogenic emissions. We found that viscosity of dry particles was in excess of the viscosity of tar pitch, which has implications for the slow rate of chemical processes in SOA. The measured viscosities were consistent with predictions based on the parametrizations developed by the Shiraiwa group. These results have implications for predicting the cloud nucleating properties of anthropogenic SOA since the presence of an organic-rich outer phase at high-RH values can lower the supersaturation with respect to water required for cloud droplet formation.
In publication [163], we reported measurements of humidity-dependent viscosity of SOA from ozonolysis of β-caryophyllene, which was an important result as the information on viscosity of SOA from sesquiterpenes is still limited. Based on the measured viscosities we demonstrated that these particles should be well-mixed under most conditions in the lower atmosphere. In publication [164], we examined viscosity and phase state in SOA produced from synthetic mixtures of volatiles emitted by healthy and stressed plants. We showed that SOA particles produced from these mixtures are much more viscous than SOA produced from a single monoterpene a-pinene, and they stay in the liquid-liquid phase separated state over a wider range of relative humidity. As a result of high viscosity, the mixing of molecules in particles emitted by stressed trees takes hours, thus affecting the dynamics of particle growth and various important properties of the resulting particles including their ability to act as cloud and ice nuclei. Based on these results, publication [169] developed a parameterization for viscosity as a function of relative humidity and temperature for pine tree SOA, and used it to predict the global distributions of SOA phase state and mixing times. We showed that the mixing times of organic molecules within SOA can often exceed one hour throughout most of the free troposphere, whereas in most of the planetary boundary layer, the glassy state is not important. We also examined chemical composition and viscosity of secondary organic aerosol (SOA) generated from real healthy and aphid-stressed Canary Island pine trees [185]. Actual trees were hauled from the greenhouse into our lab, and used as sources of tree volatiles for smog chamber experiments. The most significant result was that the aphid-stressed Canary Island pine SOA particles were consistently more viscous than healthy Canary Island pine SOA particles. The increased viscosity for the aphid-stressed pine tree SOA was attributed to the increased fraction of sesquiterpenes in the emission profile. The real pine SOA particles, both healthy and aphid-stressed, were more viscous than a-pinene SOA particles, demonstrating a significant limitation of using a single monoterpene as a model compound to predict the physicochemical properties of real biogenic SOA.
Novel Methods for Organic Aerosol Analysis
We have worked on the development of new methods designed to make HRMS analysis more sensitive, quantitative, selective, and user-friendly. This method development work has been driven by our collaborators from Purdue University, Prof. Alexander Laskin and Prof. Julia Laskin. For example, we were the first to apply desorption electrospray ionization (DESI) [66] to SOA analysis, which made it possible to examine aerosol filter samples without prior extraction. This was followed by the development of nanospray desorption electrospray ionization (nano-DESI) by Profs. A. Laskin and J. Laskin, and first applications of nano-DESI to the analysis of laboratory [70] and field [85] samples. We were the first to apply reactive nano-DESI to SOA analysis [83], an approach that makes it possible to selectively and quantitatively observe carbonyl compounds in SOA. We suggested an original approach for an approximate quantification of concentrations of SOA extracts in HR ESI-MS without using standards [79], described a new method for doing HR ESI-MS analysis of organic aerosols using a particle-into-liquid-sampler (PILS) [67], demonstrated that HR ESI-MS is a useful method for quantitative measurements of the average O/C ratios in SOA [84], and studied solvent interference in the electrospray ionization (ESI) analysis of SOA [56]. We described applications of a powerful analytical HLPC-PDA-HRMS platform for separating molecules with HPLC, recording the absorption spectra of separated molecules with a photodiode array (PDA) detector, and determining mass-to-charge ratios for the eluted compounds with ESI-HRMS [109, 114]. We also demonstrated the importance of using more than one type of ionization methods for more comprehensive analysis of OA composition [138].
Our Current Research Tools
Aerosol Chamber. We use a 5 m3 Teflon aerosol smog chamber [64], which is housed inside a 20 m3 protective enclosure. The chamber is surrounded by UV-B lamps for photochemical generation of OH from precursors like H2O2, HONO, and RONO. The OH then reacts with the VOC of interest, making SOA. SOA is collected through denuders onto filters or impacted onto other substrates for analysis. The reaction can be conducted at different VOC and NOx concentrations. The chamber is connected to a suite of state-of-the-art instruments that help control and monitor the reaction conditions: zero-air generator, NOy monitor, O3 monitor, RH/T probe, scanning mobility particle sizer (SMPS), time-of-flight aerosol mass spectrometer (ToF-AMS), proton-transfer-reaction time-of-flight mass spectrometer (PTR-ToF-MS, see below). This chamber is our main “reactor” for making model SOA.
Aerosol Flow Reactor. In addition to static aerosol chambers, we use several flow reactors to make aerosol samples. A VOC of interest is slowly injected with a syringe pump into a flow of air through a flow tube. Ozone is added to the flow through a separate port. If needed, OH is produced in the flow tube by photolysis of ozone in presence of water vapor. The gases and particles exit the flow tube, and the remaining oxidants are stripped from the mixture with a denuder, while particles are collected with a filter or an impactor. The main advantages of flow tubes include the ability to keep the aerosol concentration constant over a significant period of time (hours) and the ability to collect large amounts of SOA material (mg quantities).
PTR-ToF-MS is a sophisticated mass spectrometer that is optimized for quantitative measurements of gas-phase alcohols, carbonyls, esters, amines, olefins, aromatic compounds, etc., with sensitivities approaching parts-per-trillion by volume. The PTR-ToF-MS instrument has sufficiently high resolving power (m/∆m = 6,000) to unambiguously identify small VOCs by their accurate molecular masses and sufficiently fast response time to monitor the reaction kinetics with 1 s time resolution. The ionization mechanism involves a charge-transfer from H3O+ onto VOCs. The VOC carbon skeleton remains intact upon the proton-transfer in most cases, which significantly simplifies the data analysis. This PTR-ToF-MS instrument is shared with other research groups in the AirUCI, an institute at UCI focusing on atmospheric science research.
HRMS. For our high resolution mass spectrometry research, we rely on Orbitrap-based instruments with high resolving power (m/∆m > 105) and a soft-ionization ion sources. Our earlier experiments were done with an instrument residing at Purdue University, in the labs of Prof. Alexander Laskin and Prof. Julia Laskin. More recently, AirUCI has purchased a similar shared instrument for AirUCI users. In addition to the traditional ESI-MS approach, we often use nano-DESI developed by the Purdue team. In collaboration with Dr. Alexander Laskin and Dr. Julia Laskin, we have also been developing a method specifically designed for a detailed analysis of “brown” aerosol. This method relies on separation of the compounds by their polarity using high performance liquid chromatography (HPLC), followed by simultaneous measurement of the UV/Vis spectrum of the eluting fractions with a photodiode array absorption detector (PDA) and the high resolution mass spectrometer. |